Sustainable population and possible standards of living
- Inclusive Society Institute
- Jan 25, 2023
- 46 min read
Updated: Jan 27, 2023

Copyright © 2023
Inclusive Society Institute PO Box 12609
Mill Street
Cape Town, 8000 South Africa 235-515 NPO All rights reserved. No part of this publication may be reproduced or transmitted in any form or by any means without the permission in writing from the Inclusive Society Institute D I S C L A I M E R
Views expressed in this report do not necessarily represent the views of
the Inclusive Society Institute or its Board or Council members.
Authors:
Anton Cartwright
Prof James Blignaut
Dr Anokhi Parikh
Content Contributors:
Prof Josephine Musango
Prof Tania Ajam
Dr Motsamai Molefe
Moderator:
Prof Zweli Ndevu
Project Manager:
Daryl Swanepoel
JANUARY 2023
Content
1. Introduction
2. Learning from the history of research on population and sustainability
2.1 Biophysical constraints
2.2 Non-biophysical constraints
2.3 Learning from the past
3. Analytical approach
3.1 Modelling carrying capacity
3.2 Model structure, variables and real-world data
3.3 Scenarios to capture the influence of political economy
4. Model results and inference
5. Influences of fertility rates and policy implications
6. Conclusion
References
Appendix A: Variables and values of input data used
Appendix B: Earth overshoot day for respective countries based on “biocapacity” models.
Cover page picture source: https://journals.openedition.org/factsreports/5650
List of Tables
Table 1: Variables and sources of data for the model
Table 2: Scenario parameters
Table 3: Carrying capacity over time across four modelled scenarios
List of Figures
Figure 1: Global population over time compared to trends of a selection of
environmental indicators, showing strong correlations but not necessarily
causality
Figure 2: Number of studies by maximum human population threshold
Figure 3: Logistic functions applied in this model to capture the idea of carrying
capacity
Figure 4: A stylised causal loop diagram illustrating a selection of the various system-
wide interactions between the population, the economy and environment
Figure 5: The land sub-modelFigure 6:The cereal production and water sub-models
Figure 7: The GDP-waste and GDP-greenhouse gas sub-models
Figure 8: Modelled global carrying capacity under different scenarios
Figure 9: Carrying capacity (S0) over time, per region
Figure 10: Modelled South Asia carrying capacity under different scenarios and
unchecked population growth
“The human question is not how many people can possibly survive […] but what kind of existence is possible for those that do"
(Frank Herbert, 1965, Dune)
1. Introduction
The past 150 years have been defined by a “Great Acceleration” – the period of rapid expansion of the “economic activity of the human enterprise” (Steffen et al., 2015). This period is associated with innovation, rapid industrial expansion, commodity extraction, unprecedented improvements in agricultural productivity with the help of inorganic fertilisers, pesticides and herbicides, and rapidly rising consumption. The same 150-year period saw the human population increase from 1.2 to 7.9 billion (World Bank Data, 2022) and a raft of environmental impacts, see Figure 1, leading in some instances to the breakdown of environmental systems (McNeil, 2000; MEA, 2005; Dasgupta et al. 2021; IPCC WG2, 2022). Earth system scientists describe this period as marking a fundamental shift from the natural variability of the Holocene (the preceding 11,700-year period) to the Anthropocene in which human activity is the dominant influence on Earth’s geology, ecosystems and climate (Stoermer and Crutzen, 2000; Pearce 2009; Smith and Zeder, 2013; McNeil and Engelke, 2016; Baskin 2020).[1]

Figure 1: Global population over time compared to trends of a selection of environmental indicators, showing strong correlations but not necessarily causality
Source: Smith et al. (2009)
People have always relied on the natural environment for food and fibre and as a sink for the by-products of their economic endeavours, and this reliance has long been the source of concern about resource scarcity, environmental integrity and the implications of environmental collapse for humanity (McNeil, 2000; Crutzen, 2002; Pearce, 2009).
As the human population breaches the 8 billion mark for the first time, and in the interests of adding a contemporary perspective to what is both a longstanding intellectual curiosity and concern, the Inclusive Society Institute (South Africa) together with the Global Challenges Foundation (Sweden) commissioned research that explores the interactions between human population, environmental sustainability and human well-being. More specifically, this study sought to answer two questions:
i. What is a sustainable human population size on Earth?
ii. What are the policy measures that influence population size and population growth
rates?
The study applied a combination of literature review and systems modelling to propose a range of estimates with respect to the Earth’s plausible “carrying capacity.”[2]
Significantly, the research took place in the wake of a 2 years and 11 months drop in life expectancy in the United States between 2019 and 2022 (CDC, 2022). Covid-19 caused at least 6.5 million deaths globally and together with a spike in “unintentional injuries” – a term that is most commonly applied to drug-related deaths – accounted for the sharpest decline in United States’ life expectancy in nearly 100 years. For some, the interconnections between growing populations, habitat destruction and the outbreak of pandemics and societal stresses have created the spectre of checks on the human population (Jones et al., 2008; Dobson et al., 2020; Gibb et al., 2020; Tollefson, 2020). While the loss of life expectancy in the United States has not yet altered global population growth, the data does provide a caution against complacency on the population issue. More specifically, global events since 2019 have rocked the sanguine complacency that has characterised some policy circles that ‘everything will be OK’ or ‘technological fixes will solve our problems’ and instead highlighted the linkages between humans, other components of the natural world, conflict, political power and disease.
The research engages this context in answering the research questions and draws the high-level conclusion that consumption, resource management and choices around economic models exert a more powerful influence on sustainability and carrying capacity than population growth.
2. Learning from the history of research on population and
sustainability
From the outset, much of the research into sustainable population size has been “politically loaded”, often harnessing very human fears about well-being and longevity (Sciubba, 2022). Proponents of this research have drawn from a combination of macro-demographic and Earth system models and micro-scale biological experiments involving pin mould in a petri dish and rabbits in a hutch, to speculate about what happens when the Earth is no longer able to supply the needs of humans. Cohen (1995) observed that the debate about sustainable population size, economic well-being and cultural values has been most fierce when scientific evidence is least available. In reality, there is no shortage of “evidence” but the idea of a “sustainable human population” comprises an “essentially contested concept” that is not well-served by dogmatic, sceptic or even eclectic framings (Garver, 1978, p.168). Contestation has not prevented assumptions regarding the relationship between population size, environmental sustainability and human well-being, being embedded in many aspects of policy, often in ways that are influential but not particularly transparent: macro-economic models rely on population growth to drive economic growth; Intergovernmental Panel on Climate Change’s (IPCC’s) Shared Socioeconomic Pathways include assumptions on population growth and emissions; migration policies are often predicated on the population that a country or its economy is assumed to be able to sustain; local and regional infrastructure planning strategies are based on future levels of population, environmental integrity and economic well-being; and personal and actuarial financial plans make assumptions about future population, economic progress and environmental integrity (Pearce, 2009; Samir and Lutz, 2014).
Some of the same assumptions feature in contemporary discourses and popular culture. In the movie Infinity Wars and Avengers: Endgame, the genocidal villain, Thanos, seeks (satirically) to halve the population to cure the world of the ills of overpopulation and resource depletion and bring stability to the remaining half of the population.
2.1 Biophysical constraints
One way of understanding different studies of the Earth’s carrying capacity involves examining the assumptions they make regarding what constrains human population. Van Leeuwenhoek, famous for having invented the microscope, relied on an estimate of ‘inhabitable land’ in the world to make one of the first documented estimates of the Earth’s human carrying capacity in 1679. Extrapolating from the population of Holland (then one million people) and applying his estimate of inhabitable land, Van Leeuwenhoek concluded that the Earth could support 13 billion people. Over a century later, Thomas Malthus focused on food availability. Based on his famous model of the differential growth rates in food and population, respectively, Malthus predicted large-scale food shortages and population checks (Malthus, 1798). While Malthus’ model did include events such as war and famine, his focus on food availability failed to anticipate the influence of agricultural innovation and birth control.[3]
Paul and Anne Ehrlich focused, similarly, on food supply. In their book The Population Bomb, they forecast large-scale starvation in the 1970s and 1980s (Ehrlich and Ehrlich, 1968). Paul Ehrlich’s subsequent work included a range of commodities, and in 1980 he engaged economist Julian Simon in a bet that the price of five commodity metals would go up in the following decade due to their scarcity. Ehrlich unambiguously lost the bet but retains that he is right about the impact of population, technology and affluence in determining environmental impact. Ehrlich’s I (impact) = P (population) * A (affluence) * T (technology) model was developed with John Holdren and is still widely cited in environmental literature, despite its lack of explanatory power (Ehrlich and Holdren, 1971; Gaffney and Steffen, 2017).
Research subsequent to the Population Bomb has considered the interaction between several constraining factors in estimating the Earth’s carrying capacity for humans. The Limits to Growth remains one of the most purchased environmental books of all time and modelled the interaction between ‘population’, ‘agricultural production’, ‘natural resources’, ‘industrial production’ and ‘pollution’ (Meadows et al., 1972). The authors concluded, "The most probable result will be a rather sudden and uncontrollable decline in both population and industrial capacity" and proceeded to argue that while technological innovation and population control could delay a collapse, only a "carefully chosen set of world policies designed to stop population growth and stabilize material consumption could avoid collapse" (Meadows et al., 1972).
Other studies have drawn on different combinations of water availability, energy, carbon, forest products, non-renewable resources, heat removal, photosynthetic capacity, and the availability of land for food production. The same studies adopt a range of techniques in estimating actual maximum population size, including spatial extrapolation, modelling of multiple regions, temporal extrapolation, actual supply of a resource, hypothetical modelling, and dynamic systems modelling (see summaries in Cohen, 1995; Jeroen et al., 2004; UNEP, 2012). Population biologist Joel Cohen posited that the nitrogen cycle, available quantities of phosphorus and climate change were most likely to provide the first binding constraints on population, but conceded that, “no one knows when or at what level peak population will be reached” (Cohen, 1995). Phosphorous, a key ingredient in plant proteins, has long been considered a potentially constraining resource, but this fear has led to the discovery of new phosphate deposits on the ocean floor (Van Vuuren et al., 2010; Edixhoven et al., 2013). Applying the Food and Agriculture Organization’s assumption that there is 1.4 billion hectares of arable land available in the world, ecologist EO Wilson estimated the maximum possible human population to be 10 billion, but contingent upon the significant rider that everyone followed a vegetarian diet and humanity adopted a “generally shared long-term environmental ethic” (Wilson, 2002).
Proponents of “planetary boundaries” define a “safe operating space” for humanity based on their assessment of the thresholds of a perturbed climate, stratospheric ozone depletion, ocean acidification, biochemical flows (phosphorous and nitrogen), land system change, atmospheric aerosol loading and biosphere integrity (Rockstrom et al., 2009). Whilst the idea of a safe operating space has been useful in highlighting choices and the interaction between social and biophysical systems, proponents of this idea have struggled with the interaction between their boundaries and the data that has emerged since 2009 (Steffen et al., 2015; Raworth, 2018).
2.2 Non-biophysical constraints
The limited predictive power of past studies linking population growth and environmental integrity has led some researchers to question the significance of the relationship (Hickel and Hallegatte, 2021). Given that per capita incomes have risen much faster than the growth in population, it has been suggested that consumption growth (and associated extraction and pollution) might be more of a threat to environmental sustainability than changes in population size (Pearce, 2009; Drupp et al., 2021). This idea is broadly supported by the “Earth Overshoot Day” evidence (Appendix B), the observation that the richest 7% of people were responsible for half the greenhouse gas emissions driving climate change in 2020, and the assessment that OECD countries have contributed 92% of the historical emissions causing climate change (Pearce, 2009; Hickel, 2020). Hickel argues: "The crisis is not being caused by human beings as such, but rather by an economic system that is organized around, and dependent on, ever-increasing levels of commodity production and consumption" (Hickel and Hallegatte, 2021:2). The same focus on extraction, consumption and pollution as the primary threat to the Earth’s carrying capacity is supported by research suggesting that a child born in the United States in the early 2000s would, under the prevailing technologies, produce a lifetime carbon footprint seven times greater than a Chinese child, 46 times that of a Pakistani child, 55 times that of an Indian child, and 86 times that of a Nigerian child (Murtaugh and Schlax, 2009).
Others have questioned the very idea of natural resource constraints, given human ingenuity and innovation. Paul Romer won a Nobel Prize for Economics, drawing on empirical evidence to show non-diminishing returns to human and institutional capital in his Endogenous Growth Theory (Romer, 1986). Where Romer’s thinking is extended to include the possibility of non-diminishing returns to ecological capital (i.e., regenerating natural systems), most biophysical constraints on carrying capacity disappear (Van den Bergh, 2011; Smulders et al., 2014; Atkinson, 2015; Hickel and Hallegatte, 2021).
Interestingly, while human population continues to grow, fertility rates are falling everywhere, leading to the suggestion that either environmental or social population checks are already in effect (UNDESA, 2017). In 2021 the growth rate was 1.1%, much lower than its peak in 1968 when it grew at 2.1%. The average number of children per woman peaked in 1950 at 5.05 and had more than halved to 2.4 by 2021 (World Bank Data, 2022). More than half the women born in 1990 in the United Kingdom and Wales, had not had children, the first generation to record this statistic (Office of National Statistics, 2021).
2.3 Learning from past research
The past 300 years of research and literature on population and sustainability reveals little certainty on global carrying capacity. It does, however, highlight the emotive nature of this research question, the importance of what is measured and the timeframes over which it is measured. Rather than converge, estimates of the human population limits have diverged as the number of studies has increased. The range of published research suggests that populations between 0.5 billion and 1 trillion could live sustainably (Figure 2). Most of the research estimates that sustainable populations would be less than 16 billion, but there is no probabilistic relationship that can be applied between the frequency of estimates and actual carrying capacity of Earth.

Figure 2: Number of studies by maximum human population threshold
Source: UNEP (2012)
Reviewing past research on this topic highlights two potential research pitfalls: difficulties in imputing the contribution of innovation and adaptive human behaviour and whether it is enhancing or undermining carrying capacity (as with Malthus), and the difficulty in making accurate assumptions regarding the ecological, social and economic thresholds that should not be breached if human populations are to be sustained.
It is equally clear that most theories of demography and the impact of human populations on sustainability involve a degree of political bias and agenda (Baskin, 2020; Sciubba, 2022). In an extreme example, Garret Hardin author of the gloomy Tragedy of the Commons, called on the equally polemical and provocative need for "lifeboat ethics" in confronting a resource-constrained world (Hardin, 1974). In Hardin’s metaphor, "Each rich nation can be seen as a lifeboat full of comparatively rich people. In the ocean outside each lifeboat swim the poor of the world, who would like to get in." If any were allowed on board, Hardin argued, everyone would drown and accordingly people in the lifeboat had a duty to their species to be selfish. What Hardin’s metaphor failed to impute was that each of the people in the lifeboat was occupying the ecological equivalent of ten places.
Gender politics forms a further deep-seated bias in a number of population studies. Not only are fertility rates directly related to women’s rights and agency within societies, but the impacts of environmental degradation are born disproportionately by women (Gifford and Comeau, 2011; Schofield and Gubbels, 2019; Walk, 2021). Understanding the options and decisions available to women is largely missing from studies of population and sustainability, an oversight that undermines the body of research.
3. Analytical approach
This study sought to learn from the history of work on this topic before applying assumptions on the relationship between population and environmental sustainability. The overarching assumptions applied are listed below in the interest of transparency, and to locate this research on the wide spectrum of thinking on this topic:
i. For the purposes of this study, the Earth is assumed to be the only planet capable of
supporting human life.
ii. Planet Earth is assumed to be an open system with abundant resources many of which
have regenerative capacity, due to incoming radiative energy from the sun. In this the
human population on Earth is unlike ‘pin mould in a petri dish’ or ‘rabbits in a hutch’ in
the experiments mentioned above.
iii. It is recognised that there are many social and ecological factors, known and unknown,
that affect the maximum possible human population size on Earth. These factors interact
with each other in ways that are difficult to observe or predict. This assumption does not
preclude sensible policy responses, but does render any population sustainability model
limited in its explanatory power.
iv. It is acknowledged that Earth’s ability to sustain human life is already under extreme
pressure. Resource extraction and consumption exceeds the regenerative capacity of
most planetary systems and numerous ecological systems are in danger of collapse.
v. Humanity is assumed to be unequivocally responsible for the prevailing environmental
crises, but human impact varies greatly depending on individual income and location.
vi. Humans have a remarkable capacity to innovate and adapt their operating systems to
meet and sustain their needs. As a result, the inclusion of different human responses to
social and environmental pressures becomes critical to estimates of sustainable
population.
vii. Population is not assumed to be the only parameter impacting on sustainability.
Consumption, governance, toxicity of industrial processes and concentrations of both
political and economic power appear to influence the environmental pressures
experienced today.
viii. If something is unsustainable, then it will stop. The working assumption of this study is
that Earth systems will continue with or without people, but that population growth will
either be curtailed within a sustainable threshold by human decisions, or checked (and
possibly decline) where a collapse of ecosystem services causes food shortages,
disease, conflict, or environmentally induced declines in fertility.[4]
3.1 Modelling carrying capacity
Systems science teaches us that problematic “overshooting” occurs when periods of rapid change confront some form of barrier or threshold and the feedback loops or corrective measures are delayed or impaired (Meadows et al., 2002). Barriers or thresholds can be comprised of time, space or constraints in resources or social capacity, and they can be absolute or relative to rates of regeneration (Meadows et al., 2010). Knowing where barriers or thresholds lie, or whether feedback loops are likely to be positive or negative, can be tricky. The book Collapse – How Societies Choose to Fail or Succeed famously documents the catastrophic consequences for civilisations in which the elite believed they could insulate themselves from the impacts of ecological degradations (Diamond, 2005). In contrast, in rural villages in Kenya, the soil erosion caused by rapid population growth catalysed the social solidarity and environmental responses that led to soil protection and higher crop yields (Tiffen et al., 1993).
In this study, the notion of ‘carrying capacity’ is used as the system threshold that should not be overshot (McGuigan, 2022). Carrying capacity refers to the maximum population size of a biological species that can be sustained by that specific environment. This study considers the Earth’s carrying capacity of humans, while recognising that the maximum size of the human population is a function of the environmental systems that interact with and support that population. The modelling of carrying capacity, denotated as “K”, involves estimating the point at which the number of births is equal to the number of deaths and (once migration has been accounted for) population is stable. In the model developed for this study, carrying capacity is assumed to be a function of:
Food calorie and nutrient production: As a basic need for all humans calories and nutrients are fundamental to life; two thirds of food calories consumed globally come from just four staple crops: wheat, maize, rice and soybean (Elbehri, 2015; Rozenberg and Hallegatte, 2015; Villarroel Walker et al., 2014; Kim et al., 2019; Queiroz et al., 2021). Demand for food doubled between 1950 and 2000 (Tilman et al., 2002) and the world must produce more food in the 40 years following 2010 than in the previous 8,000 years. Agricultural innovation has driven levels of food production not imaginable by Thomas Malthus, but this has imposed an environmental burden and has its own limits. Between 1950 and 2000, agricultural yields plateaued in Europe and the United States despite a 700-fold increase in fertilisers (Foley et al., 2005; Godfray et al., 2010), and 30-35 billion tons of topsoil is lost every year (Clay, 2011).
Water provisioning: Access to freshwater is a requirement for life and economic activity (Gleick et al., 2002). Mining, agriculture, industry and urban waste have polluted and caused salinization of the 35 million km3 of freshwater systems around the world, of which only 50% are currently used by people (Gleick and Palaniappan, 2010). Any notion of freshwater limits must factor in the rates of recharge of surface water and groundwater, respectively. There is an additional 1.4 billion km2 of sea water available. As a minimum, the World Health Organisation estimates that people require access to 50 litres of water per day to live a productive and healthy life.
Energy: Access to energy varies greatly across the world, and the model described below did not use an energy parameter, per se, but did capture CO2 as a by-product of energy, as something that has to be processed by “regulating services”. Energy availability and access are a prerequisite for livelihoods, comfort, economic development and health (IEA, IRENA, UNSD, World Bank, 2021). There are strong correlations between access to energy and well-being and the ability to cope with, and respond to, disruptions. How energy is generated, by utilities and households, holds important implications for health and environmental stability both through the pathway of indoor air pollution and the linked between energy and climate change (International Energy Agency (IEA), 2014; Castan-Broto, 2017).
Resource extraction and ecosystem destruction: The extent to which societies poison or destroy the ecological integrity that supports life, affects carrying capacity (Hallegatte et al., 2019; Rockström et al., 2017). The accumulation of harmful chemicals in the ocean, freshwater systems, soil and atmosphere, or through accelerated erosion or deforestation, all undermine carrying capacity. This parameter, itself a function of what is sometimes called an extractive economy (as opposed to a circular economy) has a negative impact on carrying capacity. Historically, the presence of these environmental “bads” (extraction and destruction) has been closely correlated with human population (Lenton et al., 2019). Foremost among the risks to ecological integrity is the risk of climate change. To have a 50% chance of limiting warming to 1.5oC, the world can emit 460 billion tCO2e from January 2021 (IPCC, 2021). There are many linkages between climate change and carrying capacity. Among the most obvious are climate-induced crop failures and droughts in China, India and North America (Caparas et al., 2021). There is further evidence, but not yet sufficiently robust to have been included in the model, that persistent environmental pollutants affect carrying capacity in direct and indirect ways. The World Health Organisation notes that increased mortality results from PM2.5 above 10 micrograms per cubic metre of air, that is urban air in many of the world’s cities. There is also growing evidence that a range of phthalates, polychlorinated dibenzo-p-dioxins (PCDDs), polychlorinated dibenzofurans (PCDFs) and, specifically, polychlorinated biphenyls (PCBs) contained in pesticides or released from badly managed landfill sites or the indiscriminate burning of plastics and industrial materials, impact male and female fertility directly.
Land: As both a source (food and ecosystem services) and a sink (for the built environment and for waste processing), land is a fundamental component of carrying capacity. While some vertical farming or cellular agriculture technologies may decouple food production from land, these technologies are not available to the majority of the world, and land for ecosystem goods and services remains essential. Land is further required as a place for cities and to accommodate the urbanisation mega-trend. Suitable or optimal urban densities depend heavily on infrastructure and governance, but some high-density cities such as Medellín are associated with high levels of sustainability (Newton et al., 2022).
It is not the case that nobody dies of hunger, disease or environmental pollution at carrying capacity, but only that these deaths together with natural deaths equate over the short term to the number of births. Equally, breaching K does not necessarily lead to a population collapse. On the contrary, the model developed for this study applies a logistic function (the Verhulst-Pearl equation[5]) to reflect a population that grows exponentially, but then stabilises around a maximum threshold (an asymptote) at the carrying capacity (Figure 3) (Cohen, 1995; Bacaër, 2011). This is in contrast to an exponential function that does not accommodate a maximum, and as such is not useful in establishing the equilibrium level of K.

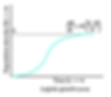
Figure 3: Logistic functions applied in this model to capture the idea of carrying capacity
Source: BioNinja[6]
To quantify the Earth’s carrying capacity, this study built and ran a model using Vensim[7] software. The model adopted a ‘systems’ approach recognising that it is functional ecosystem services – rather than a single resource – that sustain human life, and which are under critical threat. In this modelling approach, human populations are either checked or decline when ecosystems stop providing critical services. In this way, the model sought to capture the human population’s dependence on the “living fabric of ecosystems and biodiversity” (MEA, 2005; Sukhdev et al., 2014). This “fabric” is represented by four categories of critical services provided by nature, as popularised by the Millennium Ecosystem Assessment (MEA) and applied by The Economics of Ecosystems and Biodiversity (TEEB) working group, namely:
Provisioning services: such as food, freshwater, raw materials, medicinal resources
Regulating services: such as local climate and air quality, carbon sequestration and storage, extreme events, soil erosion and fertility, wastewater treatment, pollination, biological control
Cultural services: such as recreation, tourism, spiritual experiences and aesthetic appreciation
Habitat or supporting services: such as species, genetic diversity.
3.2 Model structure, variables and real-world data
No model can fully represent the extent of environmental complexity, but the idea of interacting parameters in an ecological system and interconnectedness between humans, human decisions (as shaped by both agency and culture) and environmental change, remains important to any study of carrying capacity (Sukhdev et al., 2014). The ability to capture the linkages between multiple parameters produces a very different analysis to that which would be applied if parameters were considered independently – for example, if the focus was only on food or phosphates. This is the key advantage offered by a systems model.
Selecting suitable proxies for functional ecosystem services and linking these proxies together in a manner that reflects their current real-world interdependency generates the system illustrated in Figure 4 below, complete with positive and negative feedbacks.

Figure 4: A stylised causal loop diagram illustrating a selection of the various system-wide interactions between the population, the economy and environment
The model relies on existing production modalities to establish the “direction” of the linkages between parameters, based on their positive or negative causalities. The illustration of the model in Figure 4 integrates six ‘loops’:
Loop 1 (purple): The mutual relationship between GDP and the size of the population is ambiguous, it can either be positive (reinforcing) or negative (balancing), and thus the relationship is indicated by a “?”;
Loop 2 (green): The larger the population the more land conversion takes place and the more fertilizer is used; the more that land is converted and fertiliser is used, the more extraction, solid waste, air pollution and GHG emissions as well as biodiversity impact is experienced;
Loop 3 (blue): Higher food production is linked to higher water demand, and higher water demand is positively correlated with higher environmental impact;
Loop 4 (red): Higher GDP drives higher resource and energy use, and higher resource and energy use is linked to more extraction, solid waste dumping, air pollution and GHG emissions, which are all linked to increases in the environmental impact;
Loop 5 (orange): The environmental impact is negatively correlated with population growth and GDP;
Loop 6 (black): The higher the population, the greater the land requirement, and thus the higher the environmental impact.
The model’s ‘loops’ are the product of three interlinked sub-models that describe i) land use, ii) cereal production and water use, iii) GDP-waste, GDP-greenhouse gas generation intensities, and greenhouse gas emissions.
Real-world data for land, freshwater availability, cereal production, population size and growth, and other variables, drawn from the World Bank’s World Development Indicators dataset, were used to run the sub-models (see Appendix A). The sub-models were run for each of the seven regions for which the World Bank reports data.[8] The regional disaggregation allowed the study to reflect different rates of growth, extraction and degradation, and different relationships between (for example) land and cereal production in different regions. By way of illustration, the ‘land’, ‘climate’ and ‘cereal’ sub-models are described in more detail below.
Land sub-model: While Figure 5 shows the land sub-model for East Asia and the Pacific (EAP), all sub-models were run for all seven regions. In the land sub-model, the actual land area is subdivided into five sub-categories or land-use options, namely conservation land, arable land, urban land, land for waste management, and sundry or residual land. The total available land area for each geographic area is fixed and represented by the variable “EAP area” below, but the model allows for the allocation of land across the five land-use categories to vary until the optimum at which “EAP sundry” reaches zero, at which point all other land uses are fixed for that region.
The red components of the sub-models reflect relationships between model parameters that can be adjusted by the modeller, whilst the black parameters are endogenous based on underlying formulas. The land sub-model interacts with the cereal production and water sub-models.

Figure 5: The land model
Cereal-water sub-model: The cereal production sub-model (Figure 6) interacts closely with water parameters and the land sub-model (Figure 5). Cereal production is used as a proxy for the availability of calories and nutrition. The cereal sub-model is populated with actual data for growth in cereal production in each region, but this is constrained by the water availability in that region (as determined by the water sub-model), soil erosion and climatic influences, where climate influences are determined by the emissions level in the GDP-waste and GDP-greenhouse gas sub-model (Figure 7).

Figure 6: The cereal production and water sub-models
Climate sub-model: The “GDP-waste and GDP-greenhouse gas” sub-model is also constructed for each region, using actual data for waste and emission intensities of a region, projected based on the expected economic growth of that region. Greenhouse gas emissions are linked to temperature, based on the relationship between CO2 concentrations and temperature increases.[9] Temperature is, in turn, linked to agriculture production in the cereal sub-model, to reflect the understanding that crop production is temperature dependent.

Figure 7: The GDP-waste and GDP-greenhouse gas sub-models
In each region either land, water or food becomes the binding constraint on carrying capacity, depending on whichever becomes the constraining factor first. The carrying capacity of Earth is estimated as the sum of the respective carrying capacities of the seven respective regions. This model structure includes the possibility of trade but not for migration between the regions in establishing K – given that carrying capacity is a hypothetical population number, it is independent of migration.
The list of model parameters populated by the modeller (red parameters) is provided in Table 1 with the sources of data listed. The actual values used in the base case scenario for the seven geographic regions are provided in Appendix A.

Table 1: Variables and sources of data for the model
3.3 Scenarios to capture the influence of political
economy
The baseline scenario and first model run (S0) aimed to capture the biophysical concept of carrying capacity in which the existing levels of “technically feasible” crop production efficiency, water use efficiency and land use are attained in all regions, without significant feedbacks that disturb these existing relationships. S0 relies on an indefinite continuation of the data trends between 2010–2020. There is no major climate change disruption, ecosystem collapse or outbreak of famine or disease beyond what has already been experienced in the respective regions.
S0 is important in indicating what is hypothetically possible, but does not include real-world political economy distortions that result in market failure and resource use inefficiency. Neither does it factor in disruptions to steady, linear progress. In this sense, S0 is somewhat idealistic. In reality, politics and power matters. Typically, famines are not the result of absolute food shortages, but of asymmetric power relations that block access to the available food (Sen, 1983). To reflect the influence of institutional and political-economy decisions, the model was run for three additional scenarios. The scenarios can be thought of as stories of possible future states, but they are not forecasts or predictions of the future (Rogelj, 2022). The scenarios do not reveal the likelihood of any particular future becoming reality, and it is not the case that the absence of a particular scenario means that this scenario is not possible (Huppmann et al., 2018).
The three additional scenarios applied to the running of the model are described below and the assumptions behind all four scenarios are presented in Table 2:
Scenario 1 (S1) – A resource constrained, toxic and institutionally dysfunctional world: Under this scenario dependence on natural resources continues and greenhouse gas emissions and waste per unit of productivity increase relative to S0. Negative environmental feedbacks accumulate and relative to S0, and 50% more water is required per unit of food. There is no innovation in food production per unit of land due to increasing toxicity and a lack of technology transfer to low-income countries. Energy production remains carbon intensive, as are sprawling, dysfunctional cities. To capture this plausible future, we limit the sustainable number of urban dwellers to 50 people per hectare.
Scenario 2 (S2) – A resource optimised but institutionally constrained and toxic future: The assumptions in S0 apply and food and water production efficiency improve in line with existing trends. However, under continued urbanisation and weak urban governance cities continue to sprawl, taking up valuable land and undermining technology gains. This increase is plausible in many middle-income and low-income countries, and so, implicitly, this scenario involves growing exports of food from these countries.
Scenario 3 (S3) – A resource efficient, circular economy, clean energy and institutionally functional future: In this scenario the world benefits from sustainability improvements. Greenhouse gas emissions per unit of economic productivity are 20% lower than S0, despite new sources of emissions from the oceans and permafrost. Technology gains continue to drive resource use efficiency in terms of land, water and food production, and urban governance ensures cities can accommodate 120 people per hectare in healthy, productive and long lives.
The model reflects the four scenarios by adjusting the coefficients (the red parameters) in the model. For example, while “resource use” measured by greenhouse gas emissions is always positively correlated with “waste” in the model, the extent of this correlation is higher in S1. Similarly, while food production tends to be positively correlated with fertiliser use, the extent of this correlation is much weaker in S3 than in S1. In general terms the successive scenarios S1-S3, reflect growing degrees of social and ecological sustainability in the global economic model, relative to S0.
Table 2: Scenario parameters

The study contemplated a fourth scenario (S4) involving structural (non-linear) rates of improvement in resource use efficiency and production. Under this scenario the causal relationships in Figure 4 above do not necessarily apply. It might be possible, for example, for a larger population to require less land to sustain itself or for more food to be produced while water demand goes down. This scenario is plausible if technologies such as precision fermentation and cellular agriculture – which enable food production without land – become mainstream, thereby freeing up land for the sequestration of greenhouse gases and the provision of ecosystem goods and services. Similarly, circular economies (and urban economies in particular) that produce no waste and rely almost exclusively on renewable sources, could see rising levels of GDP everywhere, with simultaneous absolute decreases in greenhouse gas emissions and other pollution. The work of ReThinkX (2021) has referenced some of the existing technologies that could support this scenario, which remains both possible and optimistic. There are, however, few reference points or data for this scenario and it is not yet possible to say how these technologies would cohere and influence societies and economies. As such, an S4 world proved difficult to capture in the model created for this study.
4. Model results and inference
Aggregating the model results from the seven regions indicates that the human population was within the Earth’s carrying capacity of 8.79 billion in 2010. Earth’s carrying capacity for humans increases under all modelled scenarios until 2050 as the benefits of existing technology manifest on food production in particular. By the end of the 21st century, global carrying capacity under the normative baseline scenario (S0) is 17,99 billion, well above the expected human population. The results are highlighted in Table 3 and Figure 8.
Under S1, negative feedback resulting from pollution, the loss of ecosystem services and diminishing returns to investment in resource extraction, begin to undermine carrying capacity from 2050 onwards, resulting in a 2100 carrying capacity of just 5.77 billion.
Under S2, in which resources accessed are used efficiently, but governance remains problematic, carrying capacity peaks in 2075 at 12.25 billion before declining to 11.63 billion by the end of the century.
Under S3, which accommodates high levels of technology innovation, well governed and compact cities and managed pollution, carrying capacity is 18.04 billion, marginally higher than in S0 due to fewer greenhouse gas emissions and climate change impacts on food production.
S4 was not modelled, for the reasons cited above, but would have produced an estimate of carrying capacity far in excess of 20 billion people.
Table 3: Carrying capacity over time across four modelled scenarios


Figure 8: Modelled global carrying capacity under different scenarios
The disaggregation of the study into regions accommodates differences in ‘start points’ in different geographies in terms of population size and growth rates, income, water-use efficiency and agricultural productivity. The regional differentiation also makes it possible to draw broad inference on the influence of inequality. It does not, however, address inequality within regions or within countries, both of which are understood to be important to the ability to forge and apply policies relating to sustainability.

Figure 9: Carrying capacity (S0) over time, per region
The model findings in the seven regions reveal that populations already exceed carrying capacity in Sub-Saharan Africa (on account of food production), South Asia (on account of food production) and MENA (on account of water scarcity). The carrying capacity of the regions under the different scenarios is shown in Figures 10-12, which also show the actual population if existing population growth were to continue. The comparison between modelled carrying capacity and actual population allow some inference on when populations have (or will) approach their carrying capacity in the respective regions.

Figure 10: Modelled South Asia carrying capacity under different scenarios and unchecked population growth
In the MENA region water constraints already exert a profound influence on carrying capacity as is indicated in Figure 9.

Figure 11: Modelled Middle East and North Africa carrying capacity under different scenarios and unchecked population growth
In Sub-Saharan Africa, food production is a constraint on the modelled growth of population and population has exceeded the region’s modelled carrying capacity since 2010. To a cursory analysis, this finding concurs with the region being a net food importer; Sub-Saharan Africa imported $43 billion worth of food in 2019. Twenty-eight (over half) of the countries in Africa received some form of food aid from the FAO in 2017 and 20% of the population experience hunger daily (Fox and Jayne, 2020). Hunger, however, is much more closely linked to food access and food distribution than food production, and links between food aid and carrying capacity are, at best, indirect. Four countries – Nigeria, Angola, Democratic Republic of Congo and Somalia – are the reason for SSA being a net food importer. Most of the other countries are food exporters, and while the value of food imports rose between 2005 and 2011, so did the value of exports as prices rose.

Figure 12: Modelled Sub-Saharan Africa carrying capacity under different scenarios and unchecked population growth
What is clear is that SSA countries have not been able to produce food at anything near their productive potential, given their land resources. The 2020 average yield in cereal production in SSA was 1.4 tons per hectare compared to the 7.2 tons per hectare averaged in North America.
Considerable scope exists for innovation, investment and technology transfers in the efforts to make the human population more sustainable. In terms of enabling countries and regions to live within their carrying capacity, it is arguably easier to address SSA’s reasons for living above its carrying capacity by increasing the region’s food production than it is to create more land for waste disposal in Europe, for example, or to decouple North America’s livelihoods from greenhouse gas emissions. In terms of carrying capacity and the social and economic stability that living within carrying capacity brings, reconfiguring the relationship between all regions’ quest for survival and the natural world that supports them, is in everyone’s interests (Robins, 2018).
Underlying the study results is the importance of how individual views on human nature influence the estimate of carrying capacity. The assumption that people are always competitive, always on a growth quest, always self-interested and acquisitive is baked into many economic and corporate cultures and strategies. Literature, from Descartes to William Golding, juxtaposes individuals against nature and against each other. It need not be this way, however. Notions of eco-civilisation and biomimicry are increasingly looking to human agency to align the direction and type of innovation and progress with nature’s systems. There are many examples in which Hardin’s notion of the tragedy of the commons has not been borne out in real life; in which environmental pressures have yielded higher levels of social cohesion and innovation (Ostrom, 1990). The potential to align with nature’s regenerative capacity holds true regardless of how the relationship between people and nature is understood – and even if you believe that ‘Nature no longer runs the Earth. We do’ (Lynas, 2011: 8) or are sceptical of technology (Baskin, 2020). Crucially, the ability to align economic endeavour with nature’s regenerative capacity – that is, move from S1 to S3 in the model – is available across a range of population sizes, suggesting that how people arrange their livelihoods and interact with the natural world is more important than population size in determining carrying capacity.
The fourth scenario (S4) involving structural (non-linear) rates of improvement in resource use efficiency and production was not modelled, for a lack of reliable reference points and data. Although the technologies required for S4 exist, it is not yet possible to say how they will be mainstreamed and influence societies and economies. What emerged in the course of the analysis, however, is that there are combinations of technology, economic activity and resource use that would see the Earth’s carrying capacity exceed 20 billion by a considerable margin. Based on existing fertility trends that suggest human population will stabilise at a maximum of 11.2 billion by 2100, humans will in no way test this carrying capacity threshold, but the thought experiment around S4 highlighted the potential for socio-technical-ecological configurations that would allow for very high living standards at high or low population levels.
5. Influences of fertility rates and policy implications
The high-level conclusion from this study argues that consumption and choices around economic and political models exert a more powerful influence (both threat and opportunity) on sustainability and carrying capacity than population growth. The scope of this work, however, included the question: What are the policy measures that influence population size and population growth rates?
Given the interest in fertility across society and politics, it is no surprise that countries have sought to intervene in population growth. The focus for sustainable population policies has previously been categorised into those that (i) “make the pie bigger” through technological innovation, (ii) “limit the number of forks” through population control and (iii) oversee “better table manners” through internalising environmental externalities and ensuring inclusive and respective “terms of interaction” (Cohen, 1995). Rationales for population control have differed, and in some instances have been fuelled by neo-colonial and racist ideologies, including the preservation of white power and access to resources in colonised countries (Kuumba, 1993; Hartmann, 1997; Folbre, 2020). After World War II the Draper Committee Report (1958) identified world population growth as a security issue for the United States and private agencies and foundations played an important role in legitimizing population control under the guise of “family planning” (Hartman, 1997).
Public resistance, most famously at the World Population Conference in Bucharest (1974), questionable impact, and the growing realisation that economic models and consumption habits exert the greatest influence on fertility rates, has not deterred the pursuit of population control measures in subsequent years. In 2019, nearly three quarters of governments that report to UNDESA had policies related to fertility: 69 had policies to lower fertility, 19 focused on maintaining current levels of fertility, and 55 aimed to raise fertility (UNDESA, 2021).
Measures focussing directly on fertility rates have historically had quite poor results. A study in the early-1990s showed that 90% of the difference in fertility rates could be attributed not to population control measures, but to women’s reported desire to have children (Pritchett et al., 1994). More specifically, contraceptive availability did not explain all changes in fertility rates, but could accelerate the declines in fertility rates once women have decided to have fewer children. This finding is supported by the fact that “wanted fertility” among women in Sub-Saharan Africa (4.2 children per women in 2017) and South-East Asia (2 children per women in 2017) has tracked actual fertility closely (World Bank Data, 2022). Accordingly, most national population policies now focus on promoting a desire for smaller families with fewer, healthier and more educated children, and delaying the age at which women have their first child through the provision of education and employment alternatives (UNDESA, 2021). The combination of these policies and social, cultural and economic conditions increasing the livelihood options available to women, has seen fertility fall in all regions and most countries. While Sub-Saharan Africa lags other regions in the world, most countries are near the end of their demographic transitions with fertility (Schoumaker, 2019) (Figure 5).

Figure 13: Fertility trends by region 2000-2020
Source: World Bank Data (accessed March 2022)
To be consistent with human rights and gender equality, population control measures need to recognise women’s right to reproductive self-determination, physical integrity and privacy, which includes the right to decide the number of children they wish to have, and the right to a full range of information and contraceptive methods. It is critical that women are able to make decisions about their fertility without fear of coercion or violence and that reproductive rights are underpinned by access to health care for women (Centre for Reproductive Rights, 2018). Top-down one-child policies or punishment for early pregnancies are not consistent with these rights and are not considered durable responses. To argue that environmental pressures alter these rights or make for an unavoidable trade-off between reproductive rights and biophysical population checks, is to miss the point that it is resource consumption and pollution in affluent countries in which fertility rates are low that is driving global environmental degradation.
It is difficult to parse the impact of population policies on reducing fertility rates relative to the impact of a country’s wider economic, social and cultural context. There is, however, some consensus on five key influences on fertility rates:
1) Investment in women’s education – There is extensive evidence that girls’/women’s education is a critical driver of fertility decline (see Bongaarts, 2020, for a summary of the literature). Murtin (2013) shows that “...average years of primary schooling among the adult population, rather than income standards, child mortality, or total mortality rates, drive fertility down by about 40% to 80% when those years grow from zero (no illiteracy) to 6 years (full literacy)” (Murtin, 2013). The causal forces for this decline are many, including a rise in the age of first marriage (Hurtich, 2017), “greater autonomy in decision making, more knowledge about the reproductive process and contraception, higher potential for earnings, and opportunity costs of childbearing” (Bongaarts, 2020).
2) Improving health systems and family planning services – Fewer deaths in childhood is a key driver of the demographic transition. An overall improvement in health systems is positively correlated with reduced child mortality, which lowers the desire for large families (World Population Review, 2019). Infant mortality has been steadily declining across the world and further declines are expected in all regions, which can be realistically expected to continue driving fertility rates down.
Family planning programs on their own tend to have a limited impact on fertility rates (Pritchett et al., 1994). However, once women want to have fewer children, family planning programs can address the unmet need for contraception and are effective in driving down wanted and unwanted fertility. While most governments have had family planning programs for several decades at this stage, the success of these programs has not been uniform (Quak and Tull, 2020). Success has been dependent on the design and implementation of programs, the availability of quality services, the flexibility of programs in adapting to local conditions, adequate monitoring and information systems, and the funding resources available (Quak and Tull, 2020). In SSA, high-quality programs in Ethiopia, Malawi and Rwanda have been associated with declines in wanted fertility, indicating that “a demographic transition can be initiated before achieving economic growth” (Bongaarts, 2020).
Although family planning services are provided for free or subsidised in public sector clinics in many countries, women continue to confront barriers to access. These include:
A lack of information on the benefits of family planning and healthy birth spacing, a lack of access to services, and a lack of method choice of contraception
Long waiting times at public facilities, high transport costs to facilities, and fears of contraceptive-related side effects
Specific barriers related to culture and family traditions faced by women and adolescents.
3) Supporting indirect policies that improve socio-economic conditions for women – The literature shows that fertility declines as poverty rates decline. Improved economic prospects tend to delay the age at which women have their first child and reduce the total number of children that women have over their lifetime. Raising women’s income, promoting policies that favour female participation in the labour force, improving land tenure for women, and social protection schemes are critical ways to achieve the reduction in fertility (Pritchett, 1994). Recent declines in fertility rates can be traced to the expansion of women’s access to paid employment. This progress has yet to be matched, however, by women’s rights to remuneration for family care from their children’s fathers and from the state (Noddings, 2002; Folbre, 2020). Historically these policies have targeted women and there is a greater recognition that policies that improve the conditions of women need to target men and boys.
4) Ensuring adequate legal frameworks to protect reproductive rights (e.g., child marriage and abortion laws) – Asymmetries in human rights lead to asymmetric economic equality, and laws and policies that codify the commitment of countries to respect and protect the rights of women are critical enablers of reduced fertility (USAID, 2013; Folbre, 2020). Legal access to abortion can reduce unwanted pregnancies in a safe manner (providing it is accompanied by quality care), yet at present, 41% of women live in countries with restrictive abortion laws.[10] Similarly, laws that increase the age of marriage or prevent child marriage can drive down fertility rates. In SSA, women who experience child marriage are eight times as likely to have more than three children, relative to women who marry after the age of 18 (Yaya, 2019). While legal frameworks are critical to protect reproductive rights, structures of patriarchal power are reinforced by their intersections and overlaps with race, citizenship and class. In this sense, improving intra-household power dynamics and reducing gender-based economy inequality has long been shown as necessary for the effective implementation of legal frameworks (De Beauvoir, 1949; Folbre, 2009).
5) Family planning campaigns – Mass media campaigns can be effective in driving demand for family planning, overcoming knowledge gaps about fertility and contraception, addressing concerns about side effects of contraception, changing norms, and increasing women’s confidence to act (USAID, 2017). Studies have shown that mass media campaigns are most effective when combined with interpersonal communication, community group engagements, and/or social marketing interventions (Quak and Tull, 2020).
6. Conclusion
This study explored the interaction between population size, environmental stability and human well-being, to establish the Earth’s human carrying capacity over the course of the 21st century and what can be done to influence it.
Estimates of maximum sustainable population size are unavoidably political, and many past studies have been used to drive religious, economic and environmental agendas that have little to do with concern for the survival of humanity. This study sought to avoid ideological biases by building and running a model that captured dynamic interactions between multiple parameters, and by not assuming ex ante that any one constraint or trend would render the human population unsustainable.
Earth’s carrying capacity for humans in 2100 ranged from 5.76 billion to 18.05 billion under the four scenarios that were modelled. To place these estimates in context, existing fertility trends make it unlikely that human population will exceed 11.2 billion by 2100, even as longevity increases significantly (UNDESA, 2017). The wide range of possible carrying capacities imply that the unambiguous answer to the research question is, ‘it depends’. Being clear on what carrying capacity depends on is critical for policy and for the harnessing of human agency in pursuit of survival and well-being.
The message from this study is that while ecological degradation presents a risk to humanity, this risk is not being driven by population growth directly. Instead, the manner in which resources are extracted, used and disposed of is more influential than absolute population size on carrying capacity and sustainability of human well-being. In this way the study challenges the assumption that current sustainability crises can be blamed on high growth populations, instead pointing to the impact of high extraction, consumption and waste populations.
The model findings further suggest that the 2010 human population appeared to be living within the planet’s carrying capacity on aggregate, but not in Sub-Saharan Africa, MENA or South Asia. This is not directly due to populations in these regions causing ecological degradation, but the distribution of technology and investment capital across the world, which ensures they do not have the agricultural productivity (or water in the case of MENA) to produce food at the levels that support their existing population.
In formulating policy responses a distinction needs to be drawn between human populations that exceed their modelled carrying capacity for that region but are not necessarily responsible for global ecological degradation, and those that are living within their region’s modelled carrying capacity but driving global environmental change through their consumption and associated extraction and pollution. This finding does not detract from the importance of technology, cultural, gender, investment and migration policies required to align economic progress with the regenerative cycles of nature (Van den Bergh, 2011; Kubiszewski et al., 2017; Schröder et al., 2020). Among other things, this requires discarding the idea of an economic ‘externality’ and adopting systems-based economies with new hybrids of indigenous knowledge and clean technology in all regions of the world to enhance the “decoupling” that appears to have commenced in some sectors and regions (Jiborn et al., 2020; Meyer and Newman, 2020; Dasgupta, 2021[11]).
Indigenous populations comprise less than 5% of the world's population and live on less than 22% of the world’s land area, but are home to 80% of the world’s biodiversity. While their level of conventional income and consumption might be below the average of populations in Europe and North America, their well-being is often slightly higher and their relationships with nature provide insights into more sustainable economic models (Reyes-Garcia et al., 2020; Abbi, 2021). Understanding how these communities co-exist with nature is one of the tasks of the Post-2020 Global Biodiversity Framework. The success of these efforts is contingent upon major economic and business institutions and financiers creating the platforms and conversation from which new economic development pathways can emerge. Based on this study, these efforts should focus on the need for change in affluent populations that currently pose the greatest threat to the environmental stability on which all humanity depends.
References
Abbi, A. 2021. Are the Jarawa India’s richest community? [Online] Available at: https://www.survivalinternational.org/not-primitive [accessed: 9 September 2022].
Atkinson, G. 2015. Natural capital and economic growth. [Online] Available at: https://assets.publishing.service.gov.uk/government/uploads/system/uploads/attachment_data/file/516948/ncc-discussion-paper-economic-growth-natural-capital.pdf [accessed: 9 September 2022].
Bacaër, N. 2011. Verhulst and the logistic equation (1838), A Short History of Mathematical Population Dynamics. [Online] Available at: https://doi.org/10.1007/978-0-85729-115-8_6
[accessed: 9 September 2022].
Baskin J. 2019. Geoengineering, the Anthropocene and the End of Nature. [Online] Available at: https://link.springer.com/book/10.1007/978-3-030-17359-3 [accessed: 9 September 2022].
Bongaarts, J. 2020. Trends in fertility and fertility preferences in Sub-Saharan Africa: the roles of education and family planning programs, Genus. [Online] Available at: https://doi.org/10.1186/s41118-020-00098-z [accessed: 9 September 2022].
Caparas, M., Castanho, A. & Schwalm, C. 2021. Increasing risks of crop failure and water scarcity in global breadbaskets by 2030, Environmental Research Letters, 16(10), 104013.
Castan-Broto, V. 2017. Energy landscapes and urban trajectories towards sustainability, Energy Policy, 108, 755-764.
Centers for Disease Control and Prevention (CDC). 2022. Life Expectancy in the U.S. Dropped for the Second Year in a Row in 2021. [Online] Available at: https://www.cdc.gov/nchs/pressroom/nchs_press_releases/2022/20220831.htm [accessed: 9 September 2022].
Center for Reproductive Rights. 2018. Gaining Ground: A Tool for Advancing Reproductive Rights Law Reform. Available at: [Online] Available at: https://reproductiverights.org/gaining-ground-a-tool-for-advancing-reproductive-rights-law-reform/ [accessed: 9 September 2022].
Clay, J. 2011. Freeze the footprint of food, Nature, 475:287-289.
Cohen, J. 1995. How many people can the earth support? The Sciences, 35(6):18-23.
Cohen, J. 1995. Population Growth and Earth’s Carrying Capacity, Science, 269:341-346.
Cote, M. & Nightingale, A.J. 2012. Resilience thinking meets social theory: Situating social change in socio-ecological systems (SES) research, Progress in Human Geography, 36:475-489.
Creutzig, F., Erb, K-H., Haberl, H. et al. 2021. Considering sustainability thresholds for BECCS in IPCC and biodiversity assessments, GCB Bioenergy, 13(4):510-515.
Crutzen, P.J. & Stoërmer, E.F. 2000. The Anthropocene, Global Change Newsletter, 41:17-18.
Crutzen, P.J. 2002. Geology of Mankind, Nature, 415:23.
Dasgupta, P. 2021. The Economics of Biodiversity: The Dasgupta Review. [Online] Available at: https://www.gov.uk/government/publications/final-report-the-economics-of-biodiversity-the-dasgupta-review [accessed: 9 September 2022].
De Beauvoir, S. 1949. The Second Sex (translated). New York: Vintage Books.
Diamond, J. 2005. Collapse: the dozen most serious environmental problems and what we can do about them, Skeptic (Altadena, CA), 11(3):36-42.
Dobson, A. et al. 2020. Ecology and economics for pandemic prevention: Investments to prevent tropical deforestation and to limit wildlife trade will protect against future zoonosis outbreaks, Science, 369(6502): 379-381.
Drupp, M., Kornek, M., Meya, J. et al. 2021. Inequality and the Environment: The Economics of a Two-Headed Hydra. [Online] Available at: https://www.cesifo.org/en/publikationen/2021/working-paper/inequality-and-environment-economics-two-headed-hydra [accessed: 12 September 2022].
Edixhoven, J.D., Gupta, J., Savenije, H.H.G. 2013. Recent revisions of phosphate rock reserves and resources: reassuring or misleading? An in-depth literature review of global estimates of phosphate rock reserves and resources, Earth System Dynamics, 5(2): 491-507.
Ehrlich, P. 2013. The Population Bomb (1968). In: The Future of Nature. New Haven: Yale University Press.
Ehrlich, P.R. & Holdren, J.P. 1971. Impact of Population Growth: Complacency concerning this component of man's predicament is unjustified and counterproductive, Science, 171(3977): 1212-1217.
Elbehri, A. 2015. Climate change and food systems: Global assessments and implications for food security and trade. [Online] Available at: https://www.fao.org/3/i4332e/i4332e.pdf [accessed: 12 September 2022].
Folbre, N. 2009. Greed, Lust and Gender: A History of Economic Ideas. Oxford: Oxford University Press.
Folbre, N. 2020. Cooperation & Conflict in the Patriarchal Labyrinth, Daedalus, 149(1): 198-212.
Foley, J.A. et al. 2005. Global consequences of land use, Science, 309(5734): 570-574.
Fox, L. & Jayne, T.S. 2020. Unpacking the misconceptions about Africa’s food imports. [Online] Available at: https://www brookings edu/blog/africa-in-focus/2020/12/14/unpacking-the-misconceptions-about-africas-food-imports/ [accessed: 12 September 2022].
Gaffney, O. & Steffen, W. 2017. The Anthropocene Equation, The Anthropocene Review, 4: 53-61.
Garver, E. 1978. Rhetoric and Essentially Contested Arguments, Philosophy & Rhetoric, 11(3): 156-172.
Gibb, R., Redding, D.W., Chin, K.Q. et al. 2020. Zoonotic host diversity increases in human-dominated ecosystems, Nature, 584: 398-402.
Gifford, R. & Comeau, L.A. 2011. Message framing influences perceived climate change competence, engagement, and behavioral intentions, Global Environmental Change, 21(4): 1301-1307.
Gleick, P. & Palaniappan, M. 2010. Peak water limits to freshwater withdrawal and use, PNAS, 107(25): 11155-62.
Gleick, P., Wolff, G., Chalecki, E. & Reyes, R. 2002. The New Economy of Water: The Risks and Benefits of Globalization and Privatization of Fresh Water. Oakland, CA: Pacific Institute.
Godfray, H.C.J., Beddington, J.R., Crute, I.R. et al. 2010. Food security: the challenge of feeding 9 billion people, Science, 327(5967): 812-818.
Hallegatte, S., Rentschler, J. & Rozenberg, J. 2019. Lifelines: The Resilient Infrastructure Opportunity. Washington, DC: World Bank.
Hardin, G. 1974. Lifeboat ethics: the case against helping the poor, Psychology Today, September: 38.
Herbert, F. 1965. Dune. New York: Putnam Publishing.
Hertrich, V. 2017. Trends in age at marriage and the onset of fertility transition in sub‐Saharan Africa, Population and Development Review, 43(S1): 112-137.
Hickel, J. 2020. Quantifying national responsibility for climate breakdown: an equality-based attribution approach for emissions in excess of the planetary boundary, The Lancet Planetary Health, 4(9): E399-E404.
Hickel, J. & Hallegatte, S. 2021. Can we live within environmental limits and still reduce poverty? Degrowth or decoupling? Development Policy Review, 40(1): E12584.
Huppmann, D., Rogelj, J., Kriegler, E. et al. 2018. A new scenario resource for integrated 1.5 °C research, Nature Climate Change, 8: 1027-1030.
IEA, IRENA, UNSD, World Bank & WHO. 2021. Tracking SDG 7: The energy progress report. [Online] Available at: https://www.iea.org/reports/tracking-sdg7-the-energy-progress-report-2021 [accessed: 12 September 2022].
International Energy Agency (IEA). 2014. Energy in Africa today. In: World Energy Outlook 2014. [Online] Available at: https://www.oecd-ilibrary.org/energy/world-energy-outlook-2014/energy-in-africa-today_weo-2014-15-en [accessed: 12 September 2022].
IPCC. 2018. Special Report: Global Warming of 1.5°C. [Online] Available at: https://www.ipcc.ch/sr15/ [accessed: 12 September 2022].
IPCC. 2021. Climate Change 2021: The Physical Science Basis. Contribution of Working Group I to the Sixth Assessment Report of the Intergovernmental Panel on Climate Change. [Online] Available at: https://www.ipcc.ch/report/ar6/wg1/ [accessed: 12 September 2022].
IPCC. 2022. Climate Change 2022: Impacts, Adaptation and Vulnerability. Contribution of Working Group II to the Sixth Assessment Report of the Intergovernmental Panel on Climate Change. [Online] Available at: https://www.ipcc.ch/report/sixth-assessment-report-working-group-ii/ [accessed: 12 September 2022].
Jiborn, M., Kulionis, V. & Kander, A. 2020. Consumption versus Technology: Drivers of Global Carbon Emissions 2000–2014, Energies 2020, 13(2): 339.
Jones, K., Patel, N., Levy, M. et al. 2008. Global trends in emerging infectious diseases, Nature, 451: 990-993.
Kubiszewski, I., Costanza, R., Anderson, S. & Sutton, P. 2017. The future value of ecosystem services: Global scenarios and national implications, Ecosystem Services, 26: 289-301.
Kuumba, M.B. 1993. Perpetuating neo-colonialism through population control: South Africa and the United States, Africa Today, 40(3): 79-85.
Leburu, V.M., El-Halabi, S., Mokganya, L. & Mills, S.L. 2009. The Contribution of the Botswana Family Planning Program to the Largest Fertility Decline in Sub-Saharan Africa. [Online] Available at: http://www.fpconference.org/2009/media/DIR_169701/15f1ae857ca97193ffff8363ffffd524.pdf [accessed: 12 September 2022].
Leburu, V.M., El-Halabi, S., Mokganya, L. & Pritchett, L.H. 1994. Desired fertility and the impact of population policies, Population and Development Review, 20(1): 1-55.
Lenton, T.M., Rockström, J., Gaffney, O. et al. 2019. Climate tipping points—too risky to bet against, Nature, 575(7784): 592-595.
Lynas, M. 2011. The God Species: Saving the Planet in the Age of Humans. Washington, D.C.: National Geographic.
Malthus, T.R. 1872. An essay on the principle of population or a view of its past and present effects on human happiness, an inquiry into our prospects respecting the future removal or mitigation of the evils which it occasions by Rev. T.R. Malthus. London: Reeves and Turner.
McGuigan, M. 2022. How many people can Earth support? [Online] Available at: https://www.livescience.com/16493-people-planet-earth-support.html [accessed: 12 September 2022].
McNeil, J.R. & Engelke, P. 2018. The great acceleration: an environmental history of the anthropocene since 1945, Journal of Landscape Ecology, 11(2): 288.
Meadows, D., Randers, J. & Meadows, M. 1972. The limits to growth: A report for the Club of Rome’s project on the predicament of mankind, 158-175. New York: Universe Books.
Meadows, D., Randers, J. & Meadows, D. 2004. A synopsis: Limits to growth: The 30-year update. United States: Chelsea Green Publishing Company.
Meldrake, S. 2020. Entangled Life: How Fungi Make Our Worlds, Change Our Minds & Shape Our Futures. New York: Random House.
Meyer, K. & Newman, P. 2020. Planetary Accounting: Quantifying How to Live Within Planetary Limits at Different Scales of Human Activity. Singapore: Springer Nature.
Millennium Ecosystem Assessment. 2005. Ecosystems and Human Well-Being. [Online] Available at: https://www.millenniumassessment.org/documents/document.356.aspx.pdf [accessed: 12 September 2022].
Murtaugh, P. & Schlax, M. 2009. Reproduction and the carbon legacies of individuals, Global Environmental Change, 19: 14-20.
Murtin, F. 2013. Long-term determinants of the demographic transition, 1870–2000, TheReview of Economics and Statistics, 95(2): 617-631.
Newton, P.W., Newman, P.W.G., Glackin, S. & Thomson, G. 2022. Greening the Greyfields: New Models for Regenerating the Middle Suburbs of Low-Density Cities. Singapore: Palgrave Macmillan.
Noddings, N. 2002. Starting at Home: Caring and Social Policy. Los Angeles: University of California Press.
Ostrom, E. 1990. Governing the Commons: The Evolution of Institutions for Collective Action. Cambridge, UK: Cambridge University Press.
Pearce, F. 2009. Consumption Dwarfs Population as Main Environmental Threat. [Online] Available at: https://e360.yale.edu/features/consumption_dwarfs_population_as_main_environmental_threat [accessed: 12 September 2022].
Quak, E. & Tull, K. 2020. Evidence of successful interventions and policies to achieve a demographic transition in sub-Saharan Africa: Ethiopia, Rwanda, and Malawi. K4D Emerging Issues Report, 30. Brighton, UK: Institute of Development Studies.
Queiroz, C., Norström, A.V., Downing, A. et al. 2021. Investment in resilient food systems in the most vulnerable and fragile regions is critical, Nature Food, 2: 546-551.
Reyes-García, V., Gallois, S., Pyhälä, A., Díaz-Reviriego, I., Fernández-Llamazares, Á., Galbraith, E. et al. 2021. Happy just because. A cross-cultural study on subjective wellbeing in three Indigenous societies, PLoS ONE, 16(5): e0251551.
Robins, N. 2018. Financing the climate change triple jump: how to align capital with a 1.5°C world.
[Online] Available at: http://www.lse.ac.uk/GranthamInstitute/news/financing-the-climate-change-triple-jump-how-to-align-capital-with-a-1-5c-world/ [accessed: 12 September 2022].
Rockström, J., Gaffney, O., Rogelj, J. et al. 2017. A roadmap for rapid decarbonization, Science, 355(6331): 1269-1271.
Rockström, J., Steffen, W., Noone, K. et al. 2009. A safe operating space for humanity, Nature, 461: 472-475.
Rockstrom, J., Steffen, W., Noone, K. et al. 2009. Planetary boundaries: exploring the safe operating space for humanity, Ecology and Society, 14(2): 32.
Rogelj, J. 2022. How not to interpret the emissions scenarios in the IPCC report. [Online] Available at: https://www.carbonbrief.org/guest-post-how-not-to-interpret-the-emissions-scenarios-in-the-ipcc-report [accessed: 12 September 2022].
Romer, P.M. 1986. Increasing Returns and Long-Run Growth, Journal of Political Economy, 94(5): 1002-1037.
Rozenberg, J. & Hallegatte, S. 2015. The impacts of climate change on poverty in 2030 and the potential from rapid, inclusive, and climate-informed development. [Online] Available at: https://elibrary.worldbank.org/doi/abs/10.1596/1813-9450-7483 [accessed: 12 September 2022].
Samir, K. & Lutz, W. 2017. The human core of the shared socioeconomic pathways: Population scenarios by age, sex and level of education for all countries to 2100, Global Environmental Change, 42: 181-192.
Schofield, D. & Gubbels, F. 2019. Informing notions of climate change adaptation: a case study of everyday gendered realities of climate change adaptation in an informal settlement in Dar es Salaam, Environment and Urbanization, 31(1):93-114.
Schoumaker, B. 2019. Stalls in fertility transitions in sub‐Saharan Africa: Revisiting the evidence, Studies in family planning, 50(3): 257-278.
Schröder, P., Lemille, A. & Desmond, P. 2020. Making the circular economy work for human development, Resources Conservation Recycling, 156: 104686.
Sciubba, J. 2022. 8 billion and counting: how sex, death and migration shape our world. New York: W.W. Norton & Company.
Sen A. (1983). Poverty and Famines: An Essay on Entitlement and Deprivation. Oxford: Oxford University Press.
Smith, B.D. & Zeder, M.A. 2013. The onset of the Anthropocene, Anthropocene, 4: 8-13.
Smith, M., Knapp, A. & Collins, S. 2009. A framework for assessing ecosystem dynamics in response to chronic resource alterations induced by global change, Ecology, 90(12): 3279-3289.
Smulders, S. et al. 2014. Growth theory and ‘green growth’, Oxford Review of Economic Policy, 30: 423-446.
Steffen, W., Broadgate, W., Deutsch, L. et al. 2015. The trajectory of the Anthropocene: The Great Acceleration, The Anthropocene Review, 2: 81-98.
Sukhdev, P., Wittmer, H. & Miller, D. 2014. The Economics of Ecosystems and Biodiversity (TEEB): Challenges and Responses. In: Nature in the Balance: The Economics of Biodiversity. Oxford: Oxford University Press.
Tiffen, M., Mortimer, M. & Gichuki, F. 1993. More People, Less Erosion: Environmental Recovery in Kenya. Chichester: John Wiley & Sons.
Tilman, D., Cassman, K.G., Matson, P.A. et al. 2002. Agricultural sustainability and intensive production practices, Nature, 418(6898): 671-677.
Tollefson, J. 2020. Why deforestation and extinctions make pandemics more likely, Nature, 584(7820): 175-176.
UNEP. 2012. One Planet, How Many People: A Review of The Earth’s Carrying Capacity. [Online] Available at: https://na.unep.net/geas/getUNEPPageWithArticleIDScript.php?article_id=88 [accessed: 12 September 2022].
United Nations Department of Economic and Social Affairs. 2017. World population projected to reach 9.8 billion in 2050, and 11.2 billion in 2100. [Online] Available at: https://www.un.org/en/desa/world-population-projected-reach-98-billion-2050-and-112-billion-2100 [accessed: 12 September 2022].
United Nations Department of Economic and Social Affairs. 2021. World Population Policies 2021: Policies related to fertility. [Online] Available at: https://www.un.org/development/desa/pd/sites/www.un.org.development.desa.pd/files/undesa_pd_2021_wpp-fertility_policies.pdf [accessed: 12 September 2022].
United Nations General Assembly. 1987. Report of the World Commission on Environment and Development: Our Common Future. [Online] Available at: https://sustainabledevelopment.un.org/content/documents/5987our-common-future.pdf [accessed: 12 September 2022].
USAID. 2013. Comprehensive Policy Processes: The agreements that outline health goals and the actions to realize them. [Online] Available at: http://www.fphighimpactpractices.org/briefs/policy [accessed: 12 September 2022].
USAID. 2017. Mass media: Reaching audiences far and wide with messages to support healthy reproductive behaviors. [Online] Available at: https://www.fphighimpactpractices.org/briefs/mass-media [accessed: 12 September 2022].
Van den Bergh, J. 2011. Environment versus growth – A criticism of “degrowth” and a plea for “a-growth”, Ecological Economics, 70(5): 881-890.
Van Vuuren, D.P., Bouwman, A.F., Beusen, A.H.W. 2010. Phosphorus demand for the 1970–2100 period: A scenario analysis of resource depletion, Global Environmental Change, 20(3): 428-439.
Villarroel Walker, R., Beck, M.B., Hall, J.W. et al. 2014. The energy-water-food nexus: Strategic analysis of technologies for transforming the urban metabolism, Journal of Environmental Management, 141C: 104-115.
Walk, P., Braunger, I., Semb, J. et al. 2021. Strengthening gender justice in a just transition: A research agenda based on a systematic map of gender in coal transitions, Energies, 14: 5985.
Wilkinson, R.G. & Pickett, K.E. 2006. Income inequality and population health: A review and explanation of the evidence, Social Science & Medicine, 62(7): 1768-1784.
Wilson, E.O. 2002. The Future of Life. New York: Vintage Books.
World Population Review. 2019. Total fertility rate 2019. [Online] Available at: http://worldpopulationreview.com/countries/total-fertility-rate/ [accessed: 12 September 2022].
Yaya, S., Odusina, E.K. & Bishwajit, G. 2019. Prevalence of child marriage and its impact on fertility outcomes in 34 sub-Saharan African countries, BMC International Health and Human Rights, 19(1): 33.
Appendix A:
Variables and values of input data used
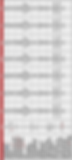
Appendix B:
Earth overshoot day for respective countries based on “biocapacity” models. The analysis suggests that extraction and consumption currently pose a greater threat to humanity living beyond the Earth’s biocapacity than population

- - - - - - - - - - - - - - - - - - - - - - - - - - - - - - - - -
[1] The Anthropocene is a contested concept. Some scientists fear that humans are the first species in the history of the planet to comprise a geo-physical source (Wilson, 2002). However, cyanobacteria and fungi have long brought about geological changes including those that gave rise to the plant kingdom (Sheldrake, 2020). Equally, notions of the Anthropocene are void of political context, and suggesting that every human is equally responsible for the environmental disruption and sustainability threat being experienced, is wrong. [2] The notion of carrying capacity was first used by engineers to describe how much cargo a ship could hold, but in the 19th century it was adopted by wildlife managers and later by ecologists (McGuigan, 2022). [3] Exponential population growth would require the growth rate to remain fixed. Instead, we have seen a rapid decline in this rate of growth over the past 70 years. For some people the jury remains out on whether Malthus was an early prophet of the broader environmental and resource crisis, and perhaps merely ‘out’ by a few hundred years with his predictions. [4] This notion of sustainability is consistent with the more common definition of sustainable development that draws from the Brundtland Commission’s 1987 report and describes, “Development that meets the needs of the present without compromising the ability of future generations to meet their own needs” (United Nations General Assembly, 1987:43). [5] https://www.toppr.com/ask/question/verhulst-pearl-is-associated-with-the-equation/ [6] https://ib.bioninja.com.au/options/option-c-ecology-and-conser/c5-population-ecology/sigmoid-growth-curve.html [7] https://vensim.com/ [8] East Asia and Pacific (EAP), Europe and Central Asia (ECA), Latin America and the Caribbean (LAC), Middle East and Northern Africa (MENA), North America (NA), South Asia (SA) and Sub-Saharan Africa (SSA). [9] https://osf.io/preprints/socarxiv/m4fdu/ https://svs.gsfc.nasa.gov/4110 [10] https://reproductiverights.org/maps/worlds-abortion-laws/ [11] The Dasgupta Review (2021, p. 119) makes the point that, “Well-functioning markets do not harbour externalities”.
- - - - - - - - - - - - - - - - - - - - - - - - - - - - - - - - -

This report has been published by the Inclusive Society Institute
The Inclusive Society Institute (ISI) is an autonomous and independent institution that functions independently from any other entity. It is founded for the purpose of supporting and further deepening multi-party democracy. The ISI’s work is motivated by its desire to achieve non-racialism, non-sexism, social justice and cohesion, economic development and equality in South Africa, through a value system that embodies the social and national democratic principles associated with a developmental state. It recognises that a well-functioning democracy requires well-functioning political formations that are suitably equipped and capacitated. It further acknowledges that South Africa is inextricably linked to the ever transforming and interdependent global world, which necessitates international and multilateral cooperation. As such, the ISI also seeks to achieve its ideals at a global level through cooperation with like-minded parties and organs of civil society who share its basic values. In South Africa, ISI’s ideological positioning is aligned with that of the current ruling party and others in broader society with similar ideals.
Email: info@inclusivesociety.org.za
Phone: +27 (0) 21 201 1589
Web: www.inclusivesociety.org.za